Revolutionizing Water Solutions: Advancements in Desalination and Treatment
- Komal Bhardwaj
- May 20
- 22 min read
Komal Bhardwaj
Department of Environment Science and Eng., GJU University, Hisar, Haryana, India
Ashwani Kumar
Department of Environment Science and Eng., GJU University, Hisar, Haryana, India
Nisha Boora
Department of Bioinformatics and Computational Biology, College of Biotechnology, CCS HAU, Hisar, Haryana, India
Abstract
Water scarcity is a global challenge that poses significant threats to human health, food security, and economic development. Desalination and advanced water treatment technologies have emerged as crucial solutions to alleviate water scarcity by converting saltwater and impaired water into freshwater suitable for various purposes. Desalination methods, including Reverse Osmosis (RO), Multi-Stage Flash (MSF) Distillation, Multi-Effect Distillation (MED), and Electrodialysis (ED), enable the conversion of saltwater into freshwater through various processes. Advanced water treatment technologies, such as Advanced Oxidation Processes (AOPs), Membrane Filtration, Activated Carbon Filtration, and Biological Treatment, further purify water from diverse sources, including impaired water and wastewater. These technologies play a vital role in meeting the increasing demand for freshwater while improving water quality. This chapter provides an overview of the principles, methods, and applications of desalination and advanced water treatment, highlighting their significance in addressing water scarcity and promoting sustainability.
I. Introduction
Water scarcity is a pressing global issue, with many regions facing limited access to freshwater resources. In a world facing an ever-growing water scarcity crisis, the significance of desalination and advanced water treatment technologies has never been more profound. As the demand for freshwater continues to outstrip its supply, exacerbated by population growth, urbanization, climate change, and pollution, our traditional water resources are becoming increasingly insufficient. In this critical juncture, the innovative approach of desalination and advanced water treatment offers a glimmer of hope and an opportunity to tackle this pressing global challenge [1].
Desalination technologies offer a viable solution by converting saltwater or brackish water into freshwater suitable for various purposes. In this section, we will explore the different desalination technologies, their principles, processes, advantages, limitations, and applications. Desalination and advanced water treatment are technologies and processes used to convert saltwater or impaired water into freshwater suitable for various purposes, including drinking water, agriculture, and industrial applications. They play a crucial role in addressing water scarcity and providing a sustainable water supply in regions where freshwater resources are limited. Desalination techniques are not limited to a single method. Initially, the primary methods relied on distillation or thermal evaporation of seawater on a large scale. While some early distillation plants were used for desalinating brackish water, the high cost hindered widespread adoption of this method in different regions globally. However, a few exceptions existed in certain countries in the Arabian Gulf region where excess or more affordable energy was available. In the 1970s, the use of membrane technologies began to gain traction, leading to the establishment of more desalination plants. These membrane technologies were utilized for desalinating both brackish water and seawater, although they are more commonly employed for brackish water due to the cost increase associated with higher salt content in water [2,3,4].
Advanced water treatment technologies complement desalination by further purifying freshwater from various sources, including wastewater and impaired water, to meet specific quality requirements. Furthermore, advanced water treatment technologies complement desalination efforts by purifying and recycling wastewater, transforming it into a valuable resource instead of a burden on the environment. These technologies play a crucial role in enhancing water reuse and promoting the circular economy, where water is conserved and recycled to its maximum potential [2,5,6,].
In this introductory exploration of desalination and advanced water treatment, we delve into the various methods, benefits, and challenges associated with these cutting-edge technologies. From reverse osmosis and distillation to nanofiltration, each technique has its advantages and limitations, making it imperative to find the right combination of solutions tailored to specific regional needs.
A. Reverse Osmosis (RO):
Reverse osmosis is the most widely used desalination technology, accounting for a significant portion of the world's desalinated water production. It involves the use of a semi-permeable membrane that allows water molecules to pass through while rejecting dissolved salts and other impurities.
Principle: Reverse osmosis relies on the application of pressure to overcome the natural osmotic pressure and force water through the membrane. The membrane acts as a barrier, selectively allowing water molecules to pass while rejecting salts and other contaminants. The rejected salts are concentrated and discharged as brine [2,4,7].
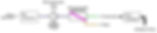
Process: The process of reverse osmosis typically involves several stages:
1. Pre-Treatment: Prior to the RO process, the feed water undergoes pre-treatment to remove suspended solids, organic matter, and other larger impurities that could foul or damage the membrane. Pre-treatment may include processes such as sedimentation, coagulation, flocculation, and filtration.
2. Pressurization: The pre-treated water is pressurized using a high-pressure pump, which forces the water through the semi-permeable membrane.
3. Separation: The pressurized water is passed through the membrane, where the salts and impurities are rejected, and the freshwater permeates through the membrane.
4. Concentration: The rejected salts and impurities accumulate as brine, which is typically discharged into the sea or a suitable disposal location.
5. Post-Treatment: The produced freshwater undergoes post-treatment to adjust its pH, mineral content, and disinfection before it is suitable for specific applications such as drinking water or industrial use [7,8,9].
Advantages:
High removal efficiency: RO technology exhibits a high removal efficiency for salts, dissolved solids, and various contaminants, resulting in high-quality freshwater.
Versatile application: RO can be applied to various water sources, including seawater, brackish water, and wastewater.
Scalability: RO plants can be designed and scaled for different capacities, ranging from small-scale systems for residential use to large municipal or industrial plants.
Process integration: RO can be integrated with other treatment technologies, such as pre-treatment processes and advanced oxidation processes, to enhance water quality.
Limitations:
Energy consumption:Â The pressurization process in RO is energy-intensive, accounting for a significant portion of the overall energy requirements. However, advancements in membrane technology and system design have led to improvements in energy efficiency.
Membrane fouling:Â RO membranes are susceptible to fouling due to the accumulation of particles, organic matter, and scaling on the membrane surface. Regular maintenance, cleaning, and periodic replacement of membranes are necessary to ensure optimal performance.
Environmental impact:Â The disposal of the concentrated brine, or reject stream, can have potential environmental impacts on marine ecosystems if not properly managed.
Initial capital cost:Â While the cost of RO systems has decreased over the years, the initial capital investment for RO plants can still be substantial, particularly for large- scale applications [10,11].
Applications:
Drinking water production: RO is widely used for producing potable water from seawater or brackish water, particularly in coastal areas where freshwater resources are scarce.
Industrial applications: RO is employed in various industries, such as power generation, food and beverage production, and semiconductor manufacturing, where high-quality process water is required.
Agriculture: RO-treated water can be used for irrigation in agriculture, allowing for cultivation in arid regions where freshwater availability is limited [2,7,12].
B. Multi-Stage Flash (MSF) Distillation:
Multi-Stage Flash distillation is a thermal desalination process that utilizes heat to evaporate seawater and subsequently condense the vapor to produce freshwater. It has been widely used in large-scale desalination plants.
Principle: MSF distillation is based on the principle of utilizing the different boiling points of water and salts to separate them.
Process: The MSF distillation process involves multiple stages, where seawater is heated in a series of flash chambers at progressively decreasing pressure. Each stage represents a lower pressure level, causing the seawater to flash into steam. The steam is then condensed, resulting in freshwater, while the remaining brine is discharged [13].
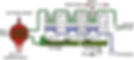
The main components of an MSF desalination plant are:
Flash chambers: These are large vessels where seawater is sprayed and heated, causing it to flash into steam. The number of flash chambers depends on the desired freshwater production capacity.
Condenser: The steam generated in the flash chambers is directed to a condenser, where it is cooled and condensed into freshwater. The condenser may use seawater, air, or another cooling medium to facilitate condensation.
Brine heater: The brine discharged from the flash chambers is heated using waste heat from the condensation process. This preheats the seawater before it enters the flash chambers, improving the overall efficiency of the system.
Evaporator tubes: These tubes carry the seawater and distribute it evenly in the flash chambers, maximizing the heat transfer and steam generation [4,6,13].
Advantages:
Proven technology: MSF distillation has a long history of successful operation in large- scale desalination plants, making it a reliable and well-established method.
Reduced fouling: MSF is less susceptible to fouling compared to membrane-based technologies, as the high temperatures involved in the process minimize the growth of organisms and deposition of solids.
Utilization of waste heat: MSF can utilize waste heat from industrial processes or power generation facilities, making it more energy-efficient and potentially reducing operational costs.
Applicable for large-scale desalination: MSF is commonly used in large-scale desalination projects where energy availability and infrastructure support its implementation.
Limitations:
Energy consumption: MSF distillation requires significant energy inputs to heat the seawater and maintain the different pressure levels in the flash chambers.
Larger footprint and infrastructure: MSF plants generally require a larger physical footprint and infrastructure compared to other desalination methods, such as reverse osmosis. This can pose challenges in densely populated or limited land areas.
Limited flexibility: MSF plants are less flexible in scaling down for smaller applications compared to membrane-based technologies, which can be more easily adjusted to varying water demands.
Environmental impact: The discharge of hot brine can have potential environmental impacts on marine ecosystems, particularly if not properly dispersed or diluted.
Applications:
Large-scale desalination projects: MSF distillation is commonly employed in large- scale desalination plants, particularly in regions with abundant heat sources and suitable infrastructure.
Power generation facilities: MSF can be integrated with power generation facilities, utilizing waste heat to drive the distillation process and produce freshwater for various uses, including cooling water supply and boiler feedwater.
Industrial applications: MSF is used in industries that require large quantities of high- quality process water, such as petrochemical refineries and power plants [14,15].
C. Multi-Effect Distillation (MED):
Principle: Multi-Effect Distillation (MED) is a thermal desalination process that utilizes multiple stages of evaporation and condensation to separate freshwater from saline water. MED operates on the principle of utilizing the heat from one stage to drive the evaporation of the next stage, maximizing energy efficiency [15].
Process: The MED process involves a series of evaporators, each operating at a progressively lower pressure and temperature. The process typically includes the following stages:
Vapor Generation: The feed water, usually seawater, enters the first evaporator (the highest-pressure stage) and is heated using external sources of energy, such as steam or hot gases. The heat causes the water to evaporate, forming water vapor.
Vapor Compression: The water vapor from the first stage enters a compressor or a series of compressors, which increase the pressure and temperature of the vapor.
Condensation: The pressurized vapor flows into the next evaporator stage, where it condenses, transferring its heat to the feed water of that stage. The condensed vapor forms freshwater, while the remaining brine is directed to subsequent stages.
Multiple Stages: The process is repeated in multiple stages, with the heat from the condensation of each stage being used to evaporate the water in the next stage. This creates a cascading effect, increasing the overall energy efficiency of the system.
Final Condensation: The last stage produces freshwater as the vapor is condensed, and the remaining brine is discharged [4,6,15].
Figure 3: Multi-Effect Distillation (MED) / Source: Mona M. Amin Abdel-Fatah and Ghada Ahmed Al Bazedi. Water Treatment and Desalination. Desalination, IntechOpen, 2020
Advantages:
Energy efficiency: MED is known for its high energy efficiency due to the utilization of waste heat or low-grade heat sources, such as industrial processes or power plants, for the evaporation process.
Flexibility: MED can handle varying feed water salinities and has the ability to adjust its operation to match the available heat sources and water demand.
Scalability: MED plants can be designed to meet a wide range of capacities, from small-scale systems to large industrial installations.
Robustness: MED is less susceptible to fouling compared to membrane-based technologies, as the high temperatures involved reduce the risk of biological growth and scaling.
Limitations:
Complexity:Â MED systems are more complex and require sophisticated control and instrumentation due to the multiple stages and the need to manage heat transfer and pressure variations.
Capital and maintenance costs:Â The initial capital investment for MED plants can be relatively high, especially for large-scale installations. Additionally, regular maintenance is required to ensure the proper functioning of the evaporators, condensers, and associated equipment.
Water recovery efficiency:Â MED systems typically have lower water recovery rates compared to membrane-based technologies like reverse osmosis.
Environmental impact:Â The disposal of the concentrated brine requires careful consideration to prevent adverse impacts on marine ecosystems.
Applications:
Industrial processes: MED is used in industries that generate excess heat or require high-quality process water, such as power generation, chemical production, and oil refineries.
Remote and off-grid areas: MED systems can be suitable for providing freshwater in remote areas where access to freshwater resources and energy infrastructure is limited.
Hybrid systems: MED can be integrated with other desalination technologies, such as RO, to optimize energy consumption and water recovery rates [15,16,17].
D. Electrodialysis (ED):
Principle: Electrodialysis (ED) is a desalination technology that utilizes an electric field to selectively remove ions from saline water. It operates on the principle of ion exchange through ion-selective membranes, which allow the passage of specific ions while blocking others.
Process: The ED process involves the following key components:
Stack: ED systems consist of a series of alternating cation-exchange and anion- exchange membranes, forming a stack. The membranes are selective and allow the passage of either positive or negative ions, while blocking the opposite charge.
Feed Water and Electrodes: The saline water, typically seawater or brackish water, is fed into the stack. Electrodes are placed at the ends of the stack and connected to a power source to generate an electric field.
Ion Migration: When the electric field is applied, positive ions (cations) migrate through the cation-exchange membranes, while negative ions (anions) migrate through the anion-exchange membranes. The selective membranes effectively separate the ions from the feed water.
Concentrate and Product Streams: The separated ions form concentrates streams, while the purified water with reduced ion content is collected as the product stream [17,18].
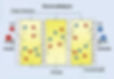
Advantages:
Energy efficiency: ED systems typically require lower energy inputs compared to thermal desalination methods, such as MSF and MED.
Chemical-free process: ED does not require the use of chemicals for ion removal, making it a chemical-free desalination option.
Scalability: ED technology can be scaled up or down to accommodate different water treatment capacities, from small-scale systems for households to large-scale installations.
Suitable for brackish water: ED is particularly suitable for desalinating brackish water sources, where the salt concentration is lower compared to seawater.
Limitations:
Membrane fouling: ED membranes can be prone to fouling due to the accumulation of ions, organic matter, and scaling. Regular cleaning and maintenance are required to ensure optimal performance.
Limited salt removal: While ED is effective in removing ions, it may not achieve the same level of salt removal as other desalination technologies like reverse osmosis.
Higher capital and maintenance costs: The initial capital investment for ED systems can be higher compared to membrane-based technologies like reverse osmosis. Additionally, membrane replacement and maintenance contribute to ongoing operational costs.
Applications:
Brackish water desalination: ED is commonly used for desalinating brackish water sources, such as groundwater and inland aquifers, to provide freshwater for agricultural, industrial, and residential purposes.
Water treatment and purification: ED can be applied in water treatment processes to remove specific ions and contaminants, improving water quality for various applications.
Resource recovery: ED can also be used for selective ion removal in resource recovery processes, such as the extraction of valuable metals or the treatment of industrial wastewater [18,19,20].
E. Advanced Oxidation Processes (AOPs):
Advanced Oxidation Processes (AOPs) are a group of water treatment techniques that employ powerful oxidation reactions to degrade and remove organic and inorganic contaminants from water. AOPs involve the generation of highly reactive hydroxyl radicals (•OH) or other oxidizing species that can effectively break down complex pollutants into simpler, less harmful compounds [6,21]. However, the common element in AOPs is the generation of highly reactive oxidants that can initiate the oxidation reactions. Examples of methods classified as AOPs:
Dark AOP
• Ozone (O3)
• Fenton (Fe2+ + H2O2)
• Electrolysis (electrodes + current)
• Sonolysis (Ultrasounds)
Light driven AOP
• Photolysis (UV + H2O2)
• Photocatalysis (light + catalyst)
• Photo-Fenton (solar light + Fenton)
a) Ozone-Based AOPs: Ozone (O3) is a strong oxidizing agent and is widely used in AOPs. The process involves the following steps:
Ozone Generation: Ozone is produced by passing oxygen gas through an electric discharge or by using an ozone generator.
Ozone Contact: The ozone is introduced into the water, either directly or as a gas or dissolved ozone bubbles, to initiate oxidation reactions.
Hydroxyl Radical Formation: Ozone reacts with water molecules, leading to the formation of hydroxyl radicals (•OH) through a process called ozone decomposition.
Oxidation Reactions: Hydroxyl radicals react with organic and inorganic contaminants in the water, breaking them down into simpler compounds through oxidation [21,22].
b) Fenton and Fenton-like Reactions: Fenton and Fenton-like reactions utilize the combination of hydrogen peroxide and ferrous ions (Fe2+) in acidic conditions. The process involves the following steps:
Addition of Ferrous Ions: Ferrous ions are added to the water along with hydrogen peroxide.
Formation of Hydroxyl Radicals: The reaction between hydrogen peroxide and ferrous ions generates hydroxyl radicals (•OH), which act as strong oxidants.
Oxidation Reactions: The hydroxyl radicals react with contaminants, initiating oxidation reactions and breaking down the complex pollutants [23].
Advantages:
Broad-spectrum treatment: AOPs are effective in the degradation and removal of a wide range of contaminants, including organic compounds, pesticides, pharmaceuticals, and some inorganic pollutants.
Rapid oxidation: AOPs generate highly reactive oxidants that can rapidly degrade pollutants, resulting in shorter treatment times compared to some other methods.
Non-selective oxidation: AOPs do not require specific knowledge of the contaminant's structure, making them suitable for treating complex mixtures of contaminants.
Versatility: AOPs can be applied to various water sources, including surface water, groundwater, and wastewater, for both drinking water treatment and industrial applications.
Limitations:
Energy Consumption: AOPs typically require energy-intensive processes for the activation of oxidizing agents or for creating the necessary conditions for oxidation reactions. The energy requirements can vary depending on the specific AOP used, such as UV lamps for photocatalysis or electrical power for ozone generation. This energy consumption adds to the operational costs of AOPs.
Cost: The cost of purchasing, installing, and maintaining the necessary infrastructure, such as ozone generators or UV reactors, can be significant. Additionally, the cost of oxidants, such as ozone or hydrogen peroxide, and any required catalysts or activating agents can add to the overall expenses.
Reaction Selectivity: While AOPs offer non-selective oxidation, targeting a wide range of contaminants, they may not effectively remove all types of pollutants. Certain compounds, such as persistent organic pollutants or refractory substances, may resist oxidation or require prolonged treatment times.
Byproduct Formation: AOPs generate reactive intermediates and byproducts during the oxidation process. These byproducts may include oxidation byproducts of the target contaminants or reaction intermediates that can be harmful or require further treatment.
Operating Conditions: AOPs often require specific operating conditions to achieve optimal performance. Factors such as pH, temperature, and contact time can influence the efficiency of the oxidation reactions. Maintaining and controlling these operating conditions can be challenging and may require additional monitoring and control systems.
Environmental Impact: The use of certain oxidizing agents, such as ozone or hydrogen peroxide, can have environmental implications. The production and transportation of these chemicals may contribute to carbon emissions and energy consumption. Additionally, the discharge of AOP-treated water into natural water bodies needs to be monitored to assess any potential impacts on aquatic ecosystems and ensure compliance with regulatory standards.
Despite these limitations, AOPs continue to be valuable tools in water treatment, particularly for the removal of complex organic compounds and recalcitrant contaminants [6, 24,25].
F. Membrane Filtration:
Membrane filtration is a widely used water treatment technology that utilizes semi-permeable membranes to separate particles, microorganisms, and dissolved substances from water. It is an effective method for producing clean and purified water for various applications, ranging from drinking water production to industrial processes. Membrane filtration operates on the principle of size exclusion, allowing the passage of water molecules while retaining contaminants based on their size and molecular properties [2,4,6].
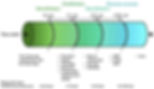
There are several types of membrane filtration processes, including:
a) Microfiltration (MF): Microfiltration membranes have relatively large pore sizes ranging from 0.1 to 10 microns. They are capable of removing suspended solids, bacteria, some viruses, and larger colloidal particles. MF is commonly used in water treatment for turbidity reduction and particle removal [6].
b) Ultrafiltration (UF): Ultrafiltration membranes have smaller pore sizes compared to MF membranes, typically ranging from 0.01 to 0.1 microns. UF membranes can remove particles, colloids, bacteria, viruses, macromolecules, and some dissolved organic compounds. UF is commonly used for the removal of pathogens, colour, and organic matter [2,10,11,12].
c) Nanofiltration (NF): Nanofiltration membranes have even smaller pore sizes, typically ranging from 0.001 to 0.01 microns. NF membranes can remove divalent ions, certain monovalent ions, organic compounds, and smaller particles. NF is often used for water softening, colour removal, and the removal of specific contaminants such as pesticides and heavy metals [26,27].
d) Reverse Osmosis (RO): Reverse osmosis membranes have the smallest pore sizes, typically less than 0.001 microns. RO membranes effectively remove salts, minerals, organic compounds, bacteria, viruses, and most dissolved substances. RO is widely used for desalination of seawater and brackish water, as well as for the production of high-quality drinking water [28,29].
Membrane Filtration Process: The membrane filtration process involves the following steps:
Pre-treatment: Prior to membrane filtration, pre-treatment steps such as coagulation, flocculation, sedimentation, and filtration may be employed to remove larger particles, suspended solids, and to improve the efficiency of the membrane system. Pre-treatment helps reduce fouling and extends the lifespan of the membranes.
Membrane Separation: The water to be treated is forced through the membrane under pressure, either in a cross-flow or dead-end configuration. As water passes through the membrane, contaminants are retained based on their size, molecular weight, and charge. Purified water, referred to as the permeate, passes through the membrane, while the rejected particles and substances form a concentrated stream, called the retentate or concentrate.
Cleaning and Maintenance: Over time, the membranes may become fouled with accumulated particles, organic matter, and scaling. Regular cleaning and maintenance procedures, such as backwashing, chemical cleaning, and membrane integrity testing, are necessary to ensure the optimal performance and longevity of the membranes [3].
Advantages of Membrane Filtration:
Effective removal of suspended solids, particles, bacteria, and viruses.
Consistent high-quality water production.
Versatile and adaptable to various water sources and contaminants.
Scalable and modular design for flexible application.
Water recovery and resource conservation.
Chemical-free operation in some cases.
Reliable and long-lasting filtration performance.
Limitations
Fouling reduces filtration efficiency.
Membrane pore size limitations may allow the passage of smaller contaminants.
Sensitivity to feed water quality affects filtration performance.
Energy-intensive process.
Disposal of concentrate generated during filtration.
Capital and operational costs.
Membrane lifespan and replacement [1,3,4].
G. Activated Carbon Filtration:
Activated carbon filtration is a process used to remove impurities, contaminants, and odors from air or water. Activated carbon is a specially treated form of carbon that has a large surface area and high adsorption capacity. It is created by heating carbon-rich materials, such as wood, coal, or coconut shells, at high temperatures in the absence of oxygen.
During the activation process, the carbon material develops a porous structure with a network of microscopic pores and a large internal surface area. These pores trap and retain various chemicals and particles through a process called adsorption. The activated carbon acts like a sponge, attracting and holding onto impurities as they pass through it. Activated carbon filters are commonly used in a variety of applications, including water treatment, air purification, and industrial processes. Here's how the filtration process typically works:
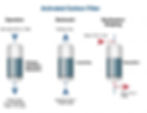
Adsorption: Contaminated air or water is passed through a bed of activated carbon. As it flows through the carbon, the impurities present in the air or water are attracted to the carbon's surface and get trapped within its pores. This includes substances like organic compounds, chlorine, volatile organic compounds (VOCs), pesticides, and certain heavy metals.
Filtration medium: Activated carbon filters can be used as standalone filters or as part of a multi-stage filtration system. In multi-stage systems, the activated carbon filter is often combined with other filter media, such as sediment filters or membrane filters, to remove larger particles and debris before the water or air reaches the carbon filter.
Contaminant removal: The activated carbon filter effectively removes impurities, contaminants, and odors from the air or water. It can improve taste and odor, remove chlorine and its byproducts, and reduce or eliminate various organic and chemical compounds.
Lifespan and maintenance: Over time, the activated carbon becomes saturated with adsorbed contaminants, reducing its effectiveness. The lifespan of the filter depends on the concentration and type of contaminants being treated. Regular maintenance, such as periodic replacement or regeneration of the activated carbon, is necessary to ensure continued filtration efficiency [30,31].
Advantages.
It is a versatile and cost-effective method for removing a wide range of impurities from both air and water.
It is widely used in residential, commercial, and industrial applications. However, it is important to note that activated carbon filters may not be effective against all types of contaminants, such as dissolved inorganic substances or certain microorganisms. Therefore, it is essential to choose the appropriate filtration system based on the specific contaminants you want to target.
Limitations:
Limited effectiveness in removing inorganic compounds and heavy metals.
Finite adsorption capacity requiring regular maintenance.
Inability to remove particulate matter without pre-filtration.
Limited effectiveness in removing microorganisms.
Flow rate reduction during filtration.
Sensitivity to extreme pH levels [6].
H. Biological treatment
Biological treatment is a process that uses living organisms, such as bacteria and other microorganisms, to degrade, transform, or remove contaminants from wastewater or polluted water sources. It is a widely used method in wastewater treatment plants and other water treatment systems. Biological treatment can be further categorized into aerobic and anaerobic processes, depending on the availability of oxygen.
a) Aerobic Biological Treatment: This process involves the use of aerobic microorganisms that require oxygen to break down organic pollutants in the water. These microorganisms convert organic matter into carbon dioxide, water, and biomass through the process of aerobic digestion. Common aerobic treatment methods include activated sludge systems, trickling filters, and sequencing batch reactors.
b) Anaerobic Biological Treatment: In contrast to aerobic treatment, anaerobic biological treatment occurs in the absence of oxygen. Anaerobic microorganisms break down organic matter through a process called anaerobic digestion, producing biogas (primarily methane and carbon dioxide) and stabilizing the wastewater. Anaerobic treatment is commonly used for high-strength organic waste, such as in anaerobic digesters for the treatment of sewage sludge or agricultural waste.
Benefits of Biological Treatment:
Effective removal of organic compounds and nutrients, reducing water pollution.
Lower energy requirements compared to some physical and chemical treatment processes.
Potential for resource recovery, such as biogas production from anaerobic digestion.
Sustainable and environmentally friendly approach utilizing natural processes.
Can be integrated with other treatment methods for comprehensive water purification.
Limitations of Biological Treatment:
Longer treatment times compared to some physical and chemical processes.
Sensitivity to fluctuations in operating conditions (e.g., temperature, pH, nutrient availability), requiring careful control.
Potential for sludge production and the need for proper sludge management.
Limited effectiveness in removing certain contaminants, such as heavy metals or certain organic compounds.
Some specific contaminants may require specialized microbial populations or additional treatment steps.
Overall, biological treatment offers an efficient and eco-friendly approach to water and wastewater treatment, but its suitability depends on the specific contaminants and treatment goals. It is often used in conjunction with other treatment methods to achieve desired water quality standards.
II. Emerging Trends and Future Developments:
Desalination technologies continue to evolve, driven by the need for more efficient and sustainable solutions. Some emerging trends and future developments include:
Emerging trends and future developments for desalination technologies and advanced treatment methods of water are focused on improving efficiency, sustainability, and the removal of emerging contaminants. Here are some key areas of advancement:
Hybrid systems: Integration of multiple desalination technologies, such as combining RO with thermal processes like MSF, to optimize energy consumption and water recovery rates.
Advanced materials: Exploring new materials for improved heat transfer, corrosion resistance, and fouling prevention to enhance the performance and durability of MED components.
Innovative heat recovery: Developing novel techniques to enhance heat recovery and optimize the utilization of waste heat from various sources, including solar energy and industrial processes.
Improving membrane performance: Developing advanced membranes with improved selectivity, fouling resistance, and durability to enhance the efficiency and lifespan of ED systems.
Energy optimization: Exploring innovative ways to optimize energy consumption in ED systems, such as integrating renewable energy sources or hybrid configurations with other desalination technologies.
Advanced system design: Enhancing system design and configuration to improve water recovery rates, minimize fouling, and reduce operational costs [2,4,5,6].
Membrane Technology: Membrane processes such as reverse osmosis (RO), nanofiltration (NF), and ultrafiltration (UF) are widely used for water treatment. The future development of membranes involves enhancing their performance, reducing fouling, and increasing their lifespan. Researchers are also exploring new types of membranes, such as graphene-based membranes, to improve water treatment efficiency.
Advanced Oxidation Processes (AOPs): Ongoing research aims to optimize AOPs by developing new catalyst materials, improving energy efficiency, and exploring novel combinations of AOPs for specific contaminants.
Removal of Emerging Contaminants: Emerging contaminants such as pharmaceuticals, microplastics, and per- and polyfluoroalkyl substances (PFAS) pose challenges to traditional treatment methods. Future developments involve developing specialized treatment processes, such as advanced oxidation, adsorption, and advanced filtration, to effectively remove these contaminants.
Smart Water Management: The integration of advanced sensors, real-time monitoring, and data analytics in water treatment systems enables better optimization, efficiency, and predictive maintenance. Smart water management systems can detect and respond to changes in water quality, optimize chemical dosing, and provide insights for process improvements.
Resource Recovery: Traditional water treatment methods often result in the generation of waste byproducts. Future developments aim to extract value from these byproducts, such as recovering energy from wastewater through anaerobic digestion or generating fertilizers from nutrient-rich sludge. This approach promotes a circular economy and improves sustainability.
III. Conclusion
Desalination and advanced water treatment technologies play a crucial role in mitigating water scarcity and providing a sustainable water supply, particularly in regions where freshwater resources are limited. These technologies have the potential to alleviate water stress and meet the growing demand for freshwater for drinking water, agriculture, and industrial applications. Desalination techniques have evolved over time, initially relying on distillation or thermal evaporation methods. However, the high cost associated with distillation hindered its widespread adoption, except in regions with excess or more affordable energy sources. The development of membrane technologies, such as Reverse Osmosis (RO), revolutionized desalination by offering a more cost-effective and energy-efficient approach. Other methods like Multi-Stage Flash (MSF) Distillation, Multi-Effect Distillation (MED), and Electrodialysis (ED) have also found their applications in desalination processes.
Furthermore, advanced water treatment technologies complement desalination by further purifying freshwater from various sources, including wastewater and impaired water. As research and development continue, there is a continuous quest to improve the efficiency, reduce energy consumption, and enhance water quality in desalination and advanced water treatment processes. By harnessing these technologies and continuing to innovate, we can work towards ensuring a sustainable and reliable freshwater supply for future generations.
IV. References
1. Curto, D.; Franzitta, V.; Guercio, A. A Review of the Water Desalination Technologies. Appl. Sci. 2021, 11, 670.
2. Mona M. Amin Abdel-Fatah and Ghada Ahmed Al Bazedi. Water Treatment and Desalination. Desalination, IntechOpen, 2020
3. Guo, L.; Xie, Y.; Sun, W.; Xu, Y.; Sun, Y. Research Progress of High-Salinity Wastewater Treatment Technology. Water 2023, 15, 684.
4.  Amit Sonune, Rupali Ghate. Developments in wastewater treatment methods, Desalination, Volume 167, 2004, Pages 55-63, ISSN 0011-9164,
5.  Yaqoob, A.A., Guerrero–Barajas, C., Ahmad, A., Ibrahim, M.N.M. and Alshammari,
M.B. (2023). Advanced Technologies for Wastewater Treatment. In Green Chemistry for Sustainable Water Purification (eds Shahid-ul-Islam, A.H. Shalla and M. Shahadat).
6.  Eckenfelder, W.W., Jr. and (2006). Wastewater Treatment. In Kirk-Othmer Encyclopedia of Chemical Technology.
7.  Blandin, G.; Verliefde, A.R.D.; Comas, J.; Rodriguez-Roda, I.; Le-Clech, P. Efficiently Combining Water Reuse and Desalination through Forward Osmosis—Reverse Osmosis (FO-RO) Hybrids: A Critical Review. Membranes 2016, 6, 37.
8.  Gu J, Liu H, Wang S, Zhang M, Liu Y. An innovative anaerobic MBR-reverse osmosis- ion exchange process for energy-efficient reclamation of municipal wastewater to NEWater-like product water. Journal of Cleaner Production. 2019;230:1287-1293.
9.  Wawrzkiewicz M, Hubicki Z. Anion exchange resins as effective sorbents for removal of acid, reactive, and direct dyes from textile wastewaters. Ion Exchange - Studies and Applications. 2015.
10. Heo J, Kim S, Her N, Park CM, Yu M, Yoon Y. Removal of contaminants of emerging concern by FO, RO, and UF membranes in water and wastewater. Contaminants of Emerging Concern in Water and Wastewater. Advanced Treatment Process 2020:139- 176.
11. Horstmeyer N, Thies C, Lippert T, Drewes JE. A hydraulically optimized fluidized bed UF membrane reactor (FB-UF-MR) for direct treatment of raw municipal wastewater to enable water reclamation with integrated energy recovery. Separation and Purification Technology. 2020;235:116165
12. Petrinic I, Korenak J, Povodnik D, Hélix-Nielsen C. A feasibility study of ultrafiltration/reverse osmosis (UF/RO)-based wastewater treatment and reuse in the metal finishing industry. Journal of Cleaner Production. 2015;101:292-300.
13. Al-Weshahi MA, Anderson A, Tian G. Exergy efficiency enhancement of MSF desalination by heat recovery from hot distillate water stages. Applied Thermal Engineering. 2013;53(2):226-233
14. Al-Weshahi MA, Tian G, Anderson A. Performance enhancement of MSF desalination by recovering stage heat from distillate water using internal heat exchanger. Energy Procedia. 2014;61:381-384.
15. Gebel J. Thermal desalination processes. In: Kucera J, editor. Desalination Water from Water. 2019:51-138. ISBN: 978-1-118-20852-6.
16. Buros, O.K. The ABCs of Desalting; International Desalination Association: Topsfield, MA, USA, 2000.
17. Shatat, M.; Riffat, S.B. Water desalination technologies utilizing conventional and renewable energy sources. Int. J. Low-Carbon Technol. 2014, 9, 1–19.
18. Nayar KG, Lienhard JHV. Brackish water desalination for greenhouse agriculture: Comparing the costs of RO, CCRO, EDR, and mono-valent-selective EDR. Desalination. 2020;475:114188.
19. Turek M, Was J, Dydo P. Brackish water desalination in RO–single pass EDR system. Desalination and Water Treatment. 2009;7(1-3):263-266.
20. Zhang Y, Liu L, Du J, Fu R, Van der Bruggen B, Zhang Y. Fracsis: Ion fractionation and metathesis by a NF-ED integrated system to improve water recovery. Journal of Membrane Science. 2017;523:385-393
21. Yang, H.; Zheng, X. Application and research progress of advanced oxidation process for degradation of organic pollutants. Technol. Water Treat. 2021, 47, 13–18.
22. Wang, R.; Zeng, D.; Yang, Y.; Qin, R.; Ke, P.; Wang, G. Research progress of fenton reagent catalytic degradation of organic wastewater. Water Wastewater Eng.2021, 464– 71.
23. Yi, B.; Yang, C.; Guo, J.; Yang, Y.; Chen, X.; Li, X. Treatment of organic wastewater with high concentration of salts using coupling process of activated carbon adsorption and fenton oxidation. Chin. J. Environ. Eng. 2013, 7, 903–907.
24. Zhou, Y.; Ji, Q.; Hu, C.; Qu, J. Recent advances in electro-oxidation technology for water treatment. J. Civ. Environ. Eng. 2022, 44, 104–118.
25. Wang, Y.; Zhang, J.; Pan, L.; Cui, F.; Zhang, B.; Yang, L. Review on research of catalytic ozonation industrial wastewater. Appl. Chem. Ind. 2019, 48, 1914–1919
26. Zahrim A, Hilal N. Treatment of highly concentrated dye solution by coagulation/flocculation–sand filtration and nanofiltration. Water Resources and Industry. 2013;3:23-34.
27. Nikbakht Fini M, Madsen HT, Muff J. The effect of water matrix, feed concentration and recovery on the rejection of pesticides using NF/RO membranes in water treatment. Separation and Purification Technology. 2019;215:521-527.
28. Mansell B, Ackman P, Tang C, Friess P, Fu P. Pilot-scale testing of a high recovery NF/RO integrated treatment system for indirect potable reuse. Proceedings of the Water Environment Federation. 2011;14:3019-3034.
29. Altaee A, Hilal N. High recovery rate NF–FO–RO hybrid system for inland brackish water treatment. Desalination. 2015;363:19-25.
30. Matilainen, A., Iivari, P., Sallanko, J., Heiska, E., & Tuhkanen, T. (2006). The Role of Ozonation and Activated Carbon Filtration in the Natural Organic Matter Removal from Drinking Water. Environmental Technology, 27(10), 1171–1180.
31. Water Environment Federation. Water Reuse, Manual of Practice SM-3, 2nd ed., Alexandria, Va., 1989.